Plankton and microbes are incredibly diverse and abundant. This group includes microorganisms, from viruses and bacteria to single-celled plants and animals, and larger animals and animal larvae that drift in the water. Bacteria, archaea (another group of simple, single-celled microbes), phytoplankton and zooplankton account for nearly 78 per cent of all marine biomass, and phytoplankton are responsible for 40 per cent of global photosynthesis.251,351 Plankton and microbes play vital roles in nutrient cycling (Section 3.3.1) and in influencing water quality, along with a multitude of ecosystem processes and symbioses (Section 3.4.6). Through their symbiotic relationships with key habitat-forming species, such as corals, sponges and algae, microbes are at the foundation of coral reefs. Plankton are the direct food source for many small fish, and invertebrates, such as mussels, clams and corals, as well as large planktivores, such as manta rays, whale sharks and baleen whales. Therefore, plankton and microbes act as key intermediaries between the physical environment and the rest of the marine food web. Some species produce toxins (for example, harmful algal blooms and box jellyfish).
Plankton and microbes are sensitive indicators of environmental change
Their global importance, short life, and sensitivity to change, make plankton and microbes indicators of ecosystem health and ecological change.352,353,354,355 Many plankton and microbial communities exhibit cyclical fluctuations in abundance, diversity and community structure on various timescales, from diurnal to seasonal, inter-annual and decadal.109,356 These fluctuations can be strongly affected by short-term environmental perturbations, such as marine heatwaves and floods.357 The timing of seasonal cycles is important for the survival of larvae of higher trophic levels, including fish. Temperature regulates plankton physiology (for example, metabolism, reproduction, ingestion) and influences species interactions, such as competition, predation and disease. Warmer temperatures alter species’ ranges (generally poleward), timing of blooms (generally earlier), diversity (higher), composition (more gelatinous and omnivorous) and individual size (smaller) 109,251 (Section 6.3.2).
One measure of the impact of temperature changes on marine life is the community temperature index (CTI), the relative abundance of species with different temperature preferences in a community.251,356 Data from the Yongala National Reference Station suggest CTI increases (indicating increasing representation of species preferring warmer waters) have occurred since 2016 for most plankton groups 251 and that this has occurred in response to continued ocean warming in the Region (Section 3.2.6). Additional data collected since 2010 at the same site indicates no change in phytoplankton CTI, but increasing community representation of zooplankton species that prefer warmer waters.250,357 Microbial abundance estimates are highly uncertain, but these groups (bacteria and archaea) have also undergone recent changes.251 In archaea communities, a stable CTI but increased species diversity suggests they might contain new species with similar temperature preferences.251 By contrast, bacterial communities have shown an increase in CTI but no increase in diversity, which suggests they do not contain new species and existing species preferring warm water are increasing in abundance.251 Longer time series are needed to confirm these trends. Additionally, the East Australian Current is bringing warm-water picoplankton (plankton less than 2 micrometres (0.002 millimetres) in size) further south, including the dinoflagellates responsible for algal blooms, which can affect food availability and toxicity for higher trophic levels.357
Box 2.5
Plankton as essential ocean variables: indicators of ecosystem health and ecological change
Plankton are excellent indicators of climate change and ecosystem health because they have short lifecycles, are ectotherms (commonly called ‘cold blooded’), and are sensitive to changes in temperature, acidity and nutrients. Phytoplankton and zooplankton biomass and diversity have been recognised as two essential ocean variables (EOVs) by the Global Ocean Observing System.362 EOVs are components critical to the functioning of marine systems and are crucial for monitoring the current state of ecosystems and how they change.
Plankton EOVs are monitored under 3 programs in the Region: the Integrated Marine Observing System (IMOS) Australian Continuous Plankton Recorder (AusCPR) survey; the IMOS Yongala National Reference Station; and the Great Barrier Reef Marine Monitoring Program (Australian Institute of Marine Science Cairns transect 363). Time-series analysis of plankton communities provides information on long‑term effects of climate variability, including at higher trophic levels, for example, changes to productivity and food security, causes and frequency of harmful algal blooms, biogeographical shifts, regime shifts, and carbon sequestration. Plankton EOVs are considered scalable, in that regional timeseries can inform understanding of the long-term effects of climate change at global scales.
Currently, sufficient sampling in time and space is a primary limitation in plankton monitoring due to the high cost of sample collection and analysis (both genomics and microscopy). Remote sensing technology, though able to estimate total phytoplankton biomass and production does not provide information on species diversity or abundance, including that of archaea, bacteria and zooplankton. Remote sensing also has limited utility in shallow coastal areas. More broadly, little is known of many plankton groups and their dynamics, as they are so diverse. Longer time‑series data and data from multiple locations are required to elucidate patterns in plankton communities over time.
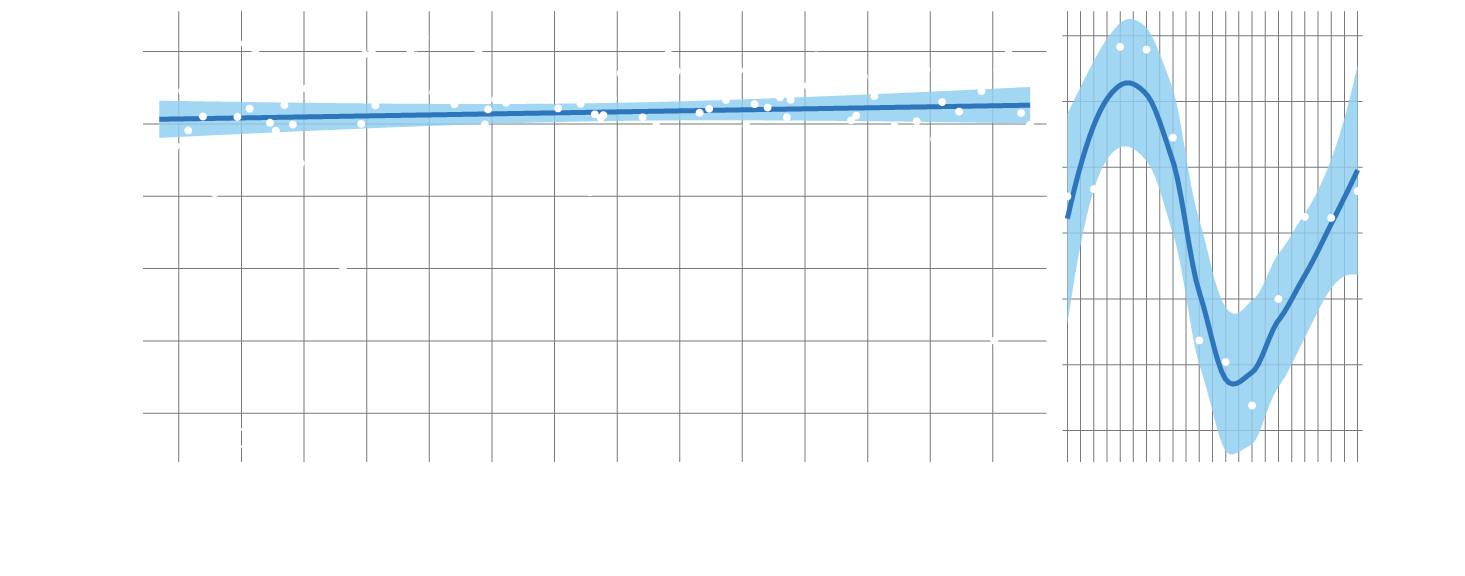
Phytoplankton abundance is predicted to decline globally under climate change because ocean stratification will reduce the availability of surface nitrate, while warmer temperatures will promote higher phytoplankton diversity.251 At the Yongala National Reference Station, no change in biomass was observed since 2010, but there was a significant increase in diversity.251 Across the Region, continuous plankton recorders measured an increase in phytoplankton biomass and an increase in diversity as might be expected from warming. Nitrogen-fixing plankton, such as Trichodesmium, exhibited a long-term increase in abundance at the Yongala station since 2010, consistent with expected patterns under climate change (Section 3.3.1).356,358 No trend was observed for the small nitrogen fixers Prochlorococcus and Synechococcus.251
Increases in zooplankton biomass have been detected in data collected at the Yongala station since 2010 and more broadly across the Region in data collected since 2014251(Box 2.5). This is consistent with the observed increase in phytoplankton and could improve feeding opportunities for invertebrates and vertebrates across the Great Barrier Reef. There was no significant change in copepod diversity, but copepod CTI has increased at the Yongala station. Zooplankton with calcareous shells, such as certain gastropods, bivalves and echinoderms, are affected by changes to ocean chemistry. A decrease in saturation of aragonite, a form of calcium carbonate (Section 6.3.2), can partially dissolve the calcium structures of these organisms, increasing maintenance costs and reducing growth rates.359 Since 2010, there has been a weakly significant decline in calcareous zooplankton abundance observed at the Yongala station. More broadly across the Region, continuous plankton recorders have revealed no significant trend, emphasising the need for longer time series data and more sampling locations.
Plankton and microbes play important roles in mitigating or exacerbating effects of climate changes on the Reef’s ecosystems.109,360 Like coral reefs (Section 3.3.1), plankton are important producers of dimethyl sulfide, which aids cloud formation, thereby reflecting solar radiation back to space. Plankton also shunt carbon from the surface to the deep ocean in a process known as the biological pump, which can lock up carbon for millennia.361 Beyond the carbon cycle, plankton are important in oceanic nitrogen, phosphorus, sulfur, and iron cycles, and in remineralisation of organic matter (Section 3.3.1).
Changes in water temperature and availability of inorganic nitrogen likely affect abundance and diversity of phytoplankton within the Region, which has trophic implications for zooplankton and beyond. Calcifying zooplankton may be affected by increasing acidification, though there is no consistent declining trend in calcifier abundance in the Region. From the limited available data, increases in diversity and abundance of warm-water species are evident across many plankton and microbe groups consistent with expectations under climate change.